Abstract
Clinical dosimetry is typically performed using ion chambers calibrated in terms of absorbed dose to water. As primary measurement standards for this quantity for low and medium energy x-rays are available only since a few years, most dosimetry protocols for this photon energy range are still based on air kerma calibration. For that reason, data for beam quality correction factors , necessary for the application of dose to water based protocols, are scarce in literature. Currently the international IAEA TRS-398 Code of Practice is under revision and new
factors for a large number of ion chambers will be introduced in the update of this protocol. Several international groups provided the IAEA with experimental and Monte Carlo based data for this revision. Within the European Community the EURAMET 16NRM03 RTNORM project was initiated for that purpose. In the present study, Monte Carlo based results for the beam quality correction factors in medium energy x-ray beams for six ion chambers applying different Monte Carlo codes are presented. Additionally, the perturbation factor pQ, necessary for the calculation of dose to water from an air kerma calibration coefficient, was determined.
The beam quality correction factor for the chambers varied in the investigated energy range by about 4%–5%, and for five out of six chambers the data could be fitted by a simple logarithmic function, if the half-value-layer was used as the beam quality specifier. Corresponding data using different Monte Carlo codes for the same ion chamber agreed within 0.5%. For the perturbation factor pQ, the data did not obey a comparable simple relationship with the beam quality specifier. The variation of pQ for all ion chambers was in the range of 3%–4%. Compared to recently published data, our pQ data is around 1% larger, although the same Monte Carlo code has been used. Compared to the latest experimental data, there are even deviations in the range of 2%.
Export citation and abstract BibTeX RIS

Original content from this work may be used under the terms of the Creative Commons Attribution 4.0 license. Any further distribution of this work must maintain attribution to the author(s) and the title of the work, journal citation and DOI.
1. Introduction
The dosimetry of medium energy x-rays poses some challenges like the non-applicability of Bragg-Gray conditions, that do not arise in high energy photon fields (Nahum 1996, Hill et al 2014). Although the international dosimetry protocol TRS-398 (Andreo et al 2001) of the IAEA introduced already in the year 2000 a dose to water based formalism for low and medium energy x-rays, there was over many years only a limited availability of standards of absorbed dose to water in the kilovoltage x-ray range. Widely used national codes of practice (CoP) like the IPEMB report (Klevenhagen et al 1996) and the AAPM TG 61 report (Ma et al 2001) did recommend an air kerma calibration. Therefore, the TRS-398 provides a description how to connect the formalism of air-kerma based CoPs with the dose to water based formalism of TRS-398.
It took several years for the national standards laboratories to develop primary measurement standards of absorbed dose to water for the medium-energy x-ray range (de Prez and de Pooter 2008, Krauss et al 2012, Rapp et al 2013, Pinto et al 2016). In 2016 a first international comparison involving four European primary standards for dose to water calibration in this photon energy range was published by Büermann et al (2016).
Monte Carlo simulations are widely used in ionization chamber dosimetry to determine correction and perturbation factors for high energy photon and proton beams. Numerous studies have investigated the detector response in high energy photon (Muir et al
2011, Tikkanen et al
2020, Andreo et al
2020) and proton (Gomà and Sterpin 2019, Baumann et al
2019) radiation fields and provided beam quality correction factors . In contrast to this, published Monte Carlo data in the low or medium x-ray energy range are scarce. Ubrich et al (2008) calculated the beam quality correction factor
of two thimble chambers in medium energy x-ray beams using Monte Carlo simulations. The authors were able to achieve good agreements between Monte Carlo based and experimental data. Chica et al (2014) did calculate the
values for the PTW 30 010 ion chamber for a large number of x-ray spectra with the Monte Carlo code PENELOPE, Andreo (2019) recently published Monte Carlo calculated, detector-independent dosimetric parameters for low- and medium-energy x-ray beams required for NK
-based dosimetry protocols. A recent study by Bancheri et al (2020) published Monte Carlo based and measured perturbation factors pQ
for the conversion of kerma based calibration coefficients into absorbed dose to water calibration coefficients for medium energy x-rays.
Currently the IAEA is working on an update of the TRS-398 CoP which will be based on the recently published ICRU Report 90 on key data for ionizing-radiation dosimetry (Seltzer et al 2016). Several international groups did provide the IAEA with experimental and Monte Carlo based data for this update. Within the European Community the EURAMET 16NRM03 RTNORM project 6 was initiated for that purpose.
In the present study the Monte Carlo based results from that consortium for six different ion chambers (PTW 30 013, PTW 31 013, PTW 31 010, NE 2571, IBA FC65-G, IBA FC65-GX) in the x-ray energy range 100–250 kV are presented. Three institutions (ENEA-INMRI: Italian National Institute of Ionizing Radiation Metrology, Rome, Italy; THM: Technische Hochschule Mittelhessen—University of Applied Sciences, Giessen, Germany and IST: Instituto Superior Técnico, Lisbon, Portugal) independently but in concert investigated the response of six ionization chambers and calculated the beam quality correction factors for the dose to water based CoP. Moreover, the necessary perturbation corrections pQ
for the conversion of kerma based calibration coefficients into absorbed dose to water were determined.
2. Materials and methods
2.1. Beam quality correction factors and perturbation factors
2.1.1. Dose to water based formalism
According to the formalism of the IAEA TRS-398 CoP (Andreo et al
2001), the reference dose Dw,Q
in z0 = 2 cm water depth for a radiation field of beam quality Q can be determined from the absorbed dose to water calibration coefficient for a medium energy x-ray reference beam quality Q0 using equation (1):

where Mw,Q
is the detector signal corrected for the influence quantities at the reference water depth z0 in a x-ray beam of quality Q and is the detector specific beam quality correction factor, accounting for the different response of the ion chamber at the beam quality Q relative to the reference beam quality Q0. By definition,
corresponds to the ratio of the calibration coefficients ND,w
for both beam qualities Q and Q0:

Equation (2) includes the assumption, that the detector signal M may be replaced by the detector dose D, i.e. . This assumption is well justified if the mean energy Wair required to generate an ion pair in air is independent from beam quality Q. According to the ICRU Report 90 (Seltzer et al
2016), the relationship between the dose to the air cavity of an ion chamber D and the chamber reading M is given by

where the constant 33.97 eV is the average energy to create an ion pair in dry air due to electrons and
is the mass of the dry air in the cavity. The correction factor kw
accounts for deviations of
from the value of 33.97 eV and the correction factor kii
corrects for the inclusion of initially ionized electrons in the cavity. According to ICRU Report 90 both correction factors are unity for primary photon energies above 100 keV, but the product
may have an impact for photon energies below 100 keV. Therefore, in preliminary investigations the impact of kw
on the beam quality correction factor
was determined. Based on the kw
data for monoenergetic photons given in ICRU 90, spectrum averaged kw,Q
data were calculated for the photon fluence spectra applied in this study (see table 1). As to be expected, the correction factor kw
was largest for the CCRI-100 spectrum, but the deviation from unity was only 2 per mille, the effect on
, i.e. the ratio
was even smaller (about 1 per mille). Moreover, according to equation (3) one has to consider the product of the two corrections
. As both corrections go in opposite directions (
) their product is closer to unity than their individual components, i.e. in any case the impact of the correction
on the beam quality correction factor
is well below one per mille and the assumption that the chamber reading M may be replaced by the dose value D is well justified even for the x-ray energies investigated here.
Table 1. Parameters of used x-ray spectra. The spectra were calculated with the software SpekCalc (Poludniowski 2007). In all cases the anode angle θ was 30° and a 50 cm air gap behind the anode was included.
![]() |
![]() | Filtration in mm | HVL | |||
---|---|---|---|---|---|---|
Spectrum | in keV | in keV | Al | Cu | Sn | in mm Cu |
CCRI-100 | 10.0 | 100 | 3.82 | — | — | 0.1461 |
CCRI-135 | 13.5 | 135 | 2.225 | 0.23 | — | 0.4708 |
CCRI-180 | 18.0 | 180 | 3.16 | 0.49 | — | 0.9863 |
CCRI-250 | 25.0 | 250 | 3.26 | 1.68 | — | 2.515 |
H-200 | 20.0 | 200 | 4.26 | 1.11 | — | 1.648 |
H-250 | 25.0 | 250 | 4.18 | 1.63 | — | 2.504 |
W-150 | 15.0 | 150 | 4.16 | — | 1.0 | 1.869 |
W-200 | 20.0 | 200 | 4.19 | — | 2.0 | 3.125 |
X-1 | 11.0 | 110 | 2.225 | — | — | 0.1062 |
X-2 | 11.0 | 110 | 2.225 | 0.0575 | — | 0.1809 |
X-3 | 11.0 | 110 | 2.225 | 0.1150 | — | 0.2485 |
X-4 | 11.0 | 110 | 2.225 | 0.1725 | — | 0.3075 |
X-5 | 11.0 | 110 | 2.225 | 0.23 | — | 0.3587 |
X-6 | 12.0 | 120 | 2.225 | 0.23 | — | 0.4030 |
2.1.2. Air-kerma based formalism
According to the TRS-398 CoP (Andreo et al 2001) the connection between air-kerma based and dose to water based formalisms for medium-energy x-rays is given as

where NK,a,Q
is the air kerma calibration coefficient free in air for the beam quality Q and is the ratio, water to air, of the mass-energy absorption coefficients, averaged for each medium over the photon energy fluence spectrum of radiation quality Q at the reference water depth z. The perturbation factor takes into account the effects on the chamber response of the difference in spectra at the chamber position between the air kerma and dose to water formalism. Furthermore, the perturbation factor accounts for the replacement of water by air and the impact of the ionization chamber material. In order to calculate the perturbation factor pQ
using Monte Carlo simulations, the ratio of the calibration coefficients ND,w,Q
and NK,a,Q
must be calculated according to the following equation:

where Ka,Q
is the air kerma free in air, Ma,Q
the signal of the detector positioned free in air for the beam quality Q and Ddet,a,Q
the absorbed dose in the sensitive volume of the detector free in air. Equation (5) is also based on the assumption, that the chamber readings Mw,Q
and Ma,Q
can be replaced by the dose values Ddet,w,Q
and Ddet,a,Q
. In a recent study Bancheri et al (2020) have shown, that the ratios of the correction factors are indeed unity for spectra in the same energy range as investigated here, i.e. the impact of the correction
on the perturbation correction pQ
is also negligible.
In summary, using equations (4) and (5), the perturbation correction pQ can be calculated by Monte Carlo simulations as follows:

2.2. Monte Carlo simulations
2.2.1. X-ray sources and reference conditions
The radiation fields of the medium energy photon beams were generated by an isotropic x-ray source emitting a limited cone beam, resulting in a circular field size of 10.5 cm in diameter at a distance of 100 cm. The spectral fluence distributions ΦE of the used beams were calculated with the software SpekCalc (Poludniowski 2007), the applied parameters are summarized in table 1. The CCRI spectra (CCEMRI 1972) were adapted by setting a suitable value for the fixed filtration thickness, so that the resulting HVL matches those of the radiation sources used by ENEA-INMRI for calibration purposes. The characteristics of the H- and W-qualities are defined according to ISO 4037-1 (1996). The spectra named X-1 to X-6 are no reference beam qualities as described by ISO or CCEMRI, they were included to fill the gap between the HVL of the CCRI-100 and CCRI-135 spectrum.
To determine the beam quality correction factors and the perturbation factors pQ
, the following dosimetric quantities were calculated: (a) the absorbed dose to water Dw
, (b) the absorbed dose to the detector's cavity in water Ddet,w,Q
and free in air Ddet,a,Q
, (c) the air kerma free in air Ka,Q
, and (d) the ratio of the mean mass energy absorption coefficients for the media water and air
at the depth of measurement z0 = 2 cm in water for quality Q.
The simulations for all ion chambers were performed under reference conditions according to the IAEA TRS-398 CoP, i.e. the source-detector distance was 100 cm. For the simulations in water, a water phantom sized 20 × 20 × 20 cm3 surrounded by air was modeled. As the x-ray spectra were calculated with a 50 cm air gap behind the anode (see table 1), the air volume in beam direction did start at 50 cm from the x-ray source (z = 0 cm), the surface of the water phantom was positioned at z = 98 cm. For the simulations free in air, the water was simply replaced by air. In all cases the field size at the detector was 10.5 cm in diameter, as also used for the measurements at ENEA-INMRI. As reference beam quality Q0 the spectrum CCRI-250 was chosen, because it is the beam quality at which primary standards of Dw realize their measurements with a relatively low uncertainty in comparison to other qualities. This holds for both water and graphite calorimeters.
2.2.2. Monte Carlo simulations settings and ion chambers
Two different Monte Carlo code systems were used by three institutions independent from each other. The EGSnrc code system (Kawrakow et al 2019a) was used by ENEA-INMRI and THM. The PENELOPE code system (Salvat 2015) was used by IST. All presented dosimetric quantities were calculated by Monte Carlo simulations applying the renormalized photoelectric cross sections and recommendations for the materials water and graphite given in the ICRU Report 90 (Seltzer et al 2016).
For the PENELOPE calculations the main program penEasy v.2015-05-13 (compatible with the PENELOPE 2014 code package) (Salvat 2015, Sempau et al 2011) was applied. The tallyEnergyDeposition counter was used to calculate the energy deposited in the chamber cavity and in water. To improve the simulation efficiency simple particle splitting was implemented for all particles (photons and electrons) entering the water volume present up to 2 cm around the chamber with a factor of 20. The transport parameters used in the PENELOPE simulations are summarized in table 2.
Table 2. Photon and electron transport parameters for the Monte Carlo simulations with PENELOPE.
Geometry region | |||
---|---|---|---|
Cavity and | Other chamber materials | Water phantom | |
bordering | and water up to 2 cm | and | |
Parameter | material | around the chamber | surrounding air |
C1 | 0 | 0.05 | 0.1 |
C2 | 0 | 0.05 | 0.1 |
Wcc (keV) | 0 | 10 | 10 |
Wcr (keV) | 0 | 1 | 1 |
Photon cutoff (keV) | 1 | 1 | 1 |
Electron cutoff (keV) | 1 | 10 | 200 |
DSMAX (cm) | 1/10 of material thickness |
For the EGSnrc simulations the code version EGSnrc 2018 was applied with the transport parameters summarized in table 3. The quantities Dw,Q , Ddet,w,Q and Ddet,a,Q were determined with the user code egs_chamber (Wulff et al 2008). The new EGSnrc application egs_kerma (Kawrakow et al 2019b) has been used to calculate kerma free in air at 100 cm source to detector distance in a cylindrical air voxel with 1 cm radius and 0.2 cm (THM) or 0.1 cm (ENEA-INMRI) height, placed symmetrically around the point of measurement. The variance reduction techniques implemented in the user code egs_chamber were used to improve the efficiency of the Monte Carlo simulations (Wulff et al 2008). The following variance reduction techniques have been used both by ENEA-INMRI and THM: intermediate phase space storage (IPSS); photon cross-section enhancement (XCSE) volume with a XCSE factor of 64 and the Russian Roulette range rejection technique with a survival probability of 1/64. The parameter ESAVE was set to 0.512 MeV.
Table 3. Photon and electron transport parameters for the Monte Carlo simulations with EGSnrc.
Transport parameter | Setting |
---|---|
Global Pcut in MeV | 0.001 |
Rayleigh scattering | On |
Photon cross sections | mcdf-xcom |
Photoelectron angular sampling | On |
Atomic relaxations | On |
Bound Compton Scattering | On |
Radiative Compton corrections | On |
Atomic relaxations | On |
Global Ecut in MeV | 0.512 |
Brems cross sections | NIST |
Brems angular sampling | KM |
Spin effects | On |
Electron impact ionization | Ik |
ESTEPE | 0.25 |
Ximax | 0.5 |
Boundary crossing algorithm | Exact |
Skin depth for BCA | 3 |
Electron-step algorithm | EGSnrc |
For both Monte Carlo codes the absorbed dose to water Dw in 2 cm water depth was calculated in a small cylindrical voxel with 1 cm radius and 0.025 cm height, placed symmetrically around the point of measurement. To calculate the detector dose Ddet , detailed models of the ionization chambers were created based on blueprints made available by the manufacturers. Monte Carlo based models of the Farmer-type chamber NE 2571 were developed independently for both Monte Carlo codes. As the chamber is not waterproof, a sleeve made of 1 mm thick PMMA was included in the simulations. The other chambers were only build for the code EGSnrc. The Farmer-type chamber PTW 30 013 was modeled independently by two partners (THM, ENEA-INMRI). Regarding the chamber FC65-G from vendor IBA, two different models were build: the type FC65-G, which is currently offered by IBA and the type FC65-GX, which is a further development of the FC65-G chamber type. Both models differ in the diameter of the central electrode, in case of the FC65-GX the diameter is about 10% larger. Moreover, the ion chambers PTW 31 010 and PTW 31 013 were included in this study. The models developed by the different partners showed some minor geometrical differences in small details like the guard ring and the stem. Exemplary cross sections of the chambers are given in figure 1.
Figure 1. Cross section of the ion chambers modeled by THM with the EGSnrc C++ class library (Kawrakow et al 2019b). The models are based on detailed blueprints from the manufacturers. The chamber FC65-GX from IBA is a further development of the chamber type FC65-G, differing in some details of the central electrode. This chamber type is not yet commercially available.
Download figure:
Standard image High-resolution image2.2.3. Calculation of mass energy absorption coefficient ratios
The mean water-to-air mass energy absorption coefficient ratios for all investigated beam qualities were calculated with EGSnrc, using two different approaches. One approach is to calculate the spectral photon fluence ΦE
at 2 cm water depth using the user code FLURZnrc and the monoenergetic mass energy absorption coefficients µen
(E)/ρ for water and air with the user code g. The mean water-to-air mass energy absorption coefficient ratio
can then be calculated as
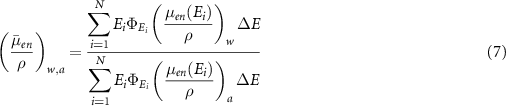
where Ei and ΔE are the photon energy and the energy bin width of the calculated spectrum, respectively.
On the other hand, the user code egs_kerma can be applied to calculate values in 2 cm water depth. Initially the user code was designed to calculate the medium kerma Km
according to the following equation:

where li
denotes the particle track length through the volume of interest V, Ei
the energy of the ith photon and n the number of photons. The user must provide data containing values for the given medium m on a logarithmic energy scale (Kawrakow et al
2019b). The individual contributions li
of photons crossing the volume of interest V are calculated by Monte Carlo simulations of the particle transport in the simulation geometry.
However, in this study egs_kerma was used to calculate the mean water-to-air mass energy absorption coefficient ratios in the volume of interest V. For that reason, kerma was calculated twice in the volume of interest: First kerma in water was calculated according to equation (8) by providing
values for water, subsequently the calculations were repeated, whereby
values for air were provided to the simulation. The ratio of both calculated kerma values results in the mean water-to-air mass energy absorption coefficient ratio
according to the following equation:
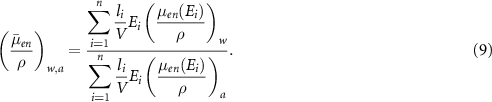
As in the first approach, the monoenergetic mass energy absorption coefficients for water and air were calculated with the user code g.
3. Results
3.1. X-ray spectra and mass energy absorption coefficients
Figure 2 shows the calculated spectral photon fluence ΦE
for the investigated beam qualities in 2 cm water depth calculated by FLURZnrc from the EGSnrc code system. In figure 3 the Monte Carlo calculated mean water-to-air mass energy absorption coefficient ratios at the same water depth as a function of the half-value layer (HVL) are shown.
values were calculated applying both approaches described in section 2.2.3. The agreement of both data sets is within 0.2%, whereat the data using the egs_kerma user code seem to be systematically higher. Additionally, the data from the TG-61 protocol and new data from the publication of Bancheri et al (2020) are included. Bancheri also used the EGSnrc system applying the FLURZnrc and g user code.
Figure 2. Spectral photon fluence ΦE of the spectra listed in table 1 at a depth of 2 cm within the water phantom, normalized to the total photon fluence at 50 cm from the radiation source.
Download figure:
Standard image High-resolution imageFigure 3. Monte Carlo calculated mean water-to-air mass energy absorption coefficient ratios at a water depth of 2 cm as a function of HVL. The dashed line is a polynomial fit to the TG-61 data. The Type-A standard uncertainty of the Monte Carlo data is smaller than 0.05%. Corresponding error bars are omitted for the sake of a clear visualization.
Download figure:
Standard image High-resolution image3.2. Beam quality correction factor
Figure 4 summarizes the calculated values of the beam quality correction factor from the absorbed dose to water based calibration protocol according to equation (2) for all investigated ionization chambers with CCRI-250 radiation quality taken as the reference quality Q0. The data are given as a function of the beam quality specifier HVL (in mm Cu). The kQ
data calculated independently by the different partners using the same Monte Carlo code (PTW 30 013) or different Monte Carlo codes for the same chamber type (NE 2571) are in good agreement (see panel (a) and (b) in figure 4). Except one data point of the NE 2571 chamber (HVL = 2.5 mm Cu), all data points agree within 0.5%. Applying the same Monte Carlo code for the same chamber type the agreement is even better.
Figure 4. Beam quality correction factor kQ,CCRI − 250 as a function of the beam quality specifier HVL for the investigated ion chambers. The dashed lines through the data points are fits, the fitting parameters are given in table 4. The type-A uncertainties of the EGSnrc Monte Carlo data are below 0.12% and are omitted for the sake of a clear visualization.
Download figure:
Standard image High-resolution imageTable 4. Parameters a and b of the applied fit function shown in figures 4 and 5. R2 is the squared correlation coefficient.
![]() | ||||||
---|---|---|---|---|---|---|
PTW 30 013 | PTW 31 013 | IBA FC65-GX | IBA FC65-G | NE 2571 | PTW 31 010 | |
THM | ENEA-INMRI | |||||
a | 1.649E−02 | 1.315E−02 | 1.531E−02 | 8.934E−03 | 1.179E−02 | |
b | 0.9856 | 0.9886 | 0.9879 | 0.9933 | 0.9905 | |
R2 | 0.9971 | 0.9982 | 0.9904 | 0.9438 | 0.9550 | |
![]() | ||||||
a | 2.2892E−02 | 2.3970E−02 | 2.6017E−02 | 2.5851E−02 | 2.0965E−02 | |
b | 1.090 | 1.0884 | 1.0897 | 1.0922 | 1.0955 | |
R2 | 0.9757 | 0.9682 | 0.9813 | 0.9629 | 0.9199 |
For the presentation of the kQ data a logarithmic scaling of the x-axis was chosen, resulting in an almost linear relationship of kQ as a function of ln(HVL). The deviations from this linear relationship are largest for the NE 2571 chamber, but except for the one data point mentioned above, the deviations are within 0.5%.
In case of the IBA FC65 chamber, the different diameter of the central electrode for the models FC65-G and FC-65GX has a clear impact. For the smallest HVL (0.1461 mm Cu), the difference between the kQ values is already in the range of 1.6%.
In comparison to all other chambers, the kQ,CCRI − 250 values for the PTW 31 010 chamber show a different relationship as a function of ln(HVL). For this chamber, a more bi-linear characteristic is present. Data points below a HVL of about 1 mm Cu obey a different slope as the data points above 1 mm HVL.
3.3. Air kerma related quantities
Figure 5 shows the ratio of the calibration coefficients ND,w,Q over NK,a,Q calculated according to equation(5) again as a function of the beam quality specifier HVL given in mm Cu. As can be seen, the deviations from a logarithmic fit are larger compared to the kQ,CCRI − 250 data. The deviations especially for the lowest beam quality are already in the range of 1%. The parameters of the fit functions shown in figure 5 are also summarized in table 4. For the PTW 30 013 chamber additionally data from the report from Burns et al (2017) are included in figure 5. These data were calculated with PENELOPE. The agreement with the EGSnrc data from this study is within 0.3%.
Figure 5. Monte Carlo calculated ratios of the calibration coefficients ND,w,Q over NK,a,Q as a function of the beam quality specifier HVL for different ion chambers. The Type-A standard uncertainty of the Monte Carlo data is in all cases smaller than 0.12%. All data except the BIPM data were calculated with EGSnrc. The BIPM data are taken from Burns et al (2017). These data were calculated with PENELOPE. The dashed lines through the data points are fits, the fitting parameters are given in table 4.
Download figure:
Standard image High-resolution imageComparing the kQ
and data of the FC65 chamber type, it is noticeable, that the large deviations present in the kQ
data (see figure 4(c)) are not present in the
data (figure 5(c)). Despite the fact, that both chambers (FC65-G and FC65-GX) differ in their central electrode diameter, the ratios
agree within 0.5%.
Regarding the perturbation factor pQ
required for the kerma based dosimetry protocols (Andreo et al
2001, Ma et al
2001), there is no simple logarithmic relationship with HVL (see figure 6). At small HVL values pQ
is about unity for all chambers, i.e. the ratio equals within 1% the mean water-to-air mass energy absorption coefficient ratios
. Up to HVL values of about 0.4–0.5 mm Cu the pQ
values for the larger Farmer-type chambers increase by about 3%. For the smaller PTW 31 010 chamber, pQ
increases by about 4%. Beyond the maximum the pQ
values decrease with HVL, reaching a value of about 1.01 for the largest HVL investigated in this study. For the chambers PTW 30 013 and NE 2571, simulated independently by THM and ENEA-INMRI, there is a good agreement between the calculated pQ
values (within 0.4%). Regarding the IBA FC65 chamber, the smaller diameter of the central electrode in the FC65-G model results in pQ
values, which are systematically about 0.4%–0.5% larger than the values for the FC65-GX model.
Figure 6. Monte Carlo calculated perturbation factors pQ as a function of the HVL for different ion chambers. The type-A standard uncertainty of the Monte Carlo data from this study (EGSnrcTHM , EGSnrcENEA ) is in all cases smaller than 0.12% and corresponds to the symbol width. The data from Bancheri are taken from Bancheri et al (2020), the TG-61 data from Ma et al (2001). The logarithmic x-axis is chosen for better visualization.
Download figure:
Standard image High-resolution imageIncluded in figure 6 are also Monte Carlo based and experimental data taken from the publication of Bancheri et al (2020). Their Monte Carlo data were also calculated with the EGSnrc code system and there are deviations to our data in the range of 1%. Their experimental data, determined at the German national metrology institute Physikalisch-Technische Bundsanstalt (PTB), are based on their water calometry-based absorbed dose to water primary standard. These data are clearly below all Monte Carlo based data, in comparison to the data of this study the deviations are already up to 2%. For the PTW 30 013 chamber additionally data based on the publication from Burns et al (2017) are shown. These data were calculated using equation (6), using the ratios given in figure 5 and the mean water-to-air mass energy absorption coefficient ratios
from this study calculated with the FLURZnrc code.
4. Discussion
The ratios of the mass energy absorption coefficients calculated on the basis of the spectral photon fluence and the monoenergetic mass energy absorption coefficients
for the CCRI- and H-qualities are in very good agreement (
0.06%) with published data from Büermann et al (2016), Andreo (2019) and Bancheri et al (2020). All these data were calculated using the same approach and the same Monte Carlo code EGSnrc. Regarding the W radiation qualities (HVL = 1.87 and 3.125 mm Cu) the agreement is worse. These W-spectra are the only ones including a Sn-filtering, resulting in a completely different shape of the spectra in comparison to the CCRI- or H-qualities, especially in the low energy region (see figure 2). The data calculated with the egs_kerma code are up to 0.2% larger then those applying the FLURZnrc code. This deviation can be ascribed to differences in the different energy binning used for the computation of the monoenergetic mass energy absorption coefficients for water and air with the user code g. The binning is done on a log scale as required for the egs_kerma code (equation (9)), while in the second case (equation (7)) a linear scale (bin width 1 keV) is used matching the binning already applied for the photon fluence calculation with the FLURZnrc code.
The ratios of the dose to water based and kerma based calibration coefficients for the chambers PTW 30 013 and NE 2571 were also independently calculated by ENEA-INMRI and THM. Both data sets agree within 0.3% (see figure 5.), the same is true for the resulting perturbation factors pQ
given in figure 6. Comparable pQ
data for the chambers PTW 30 013, NE 2571 and IBA FC65-G were recently published by Bancheri et al (2020). For the PTW 30 013 they can also be deduced from a report from Burns et al (2017). Whereas the data from Burns are based on Monte Carlo calculations with the PENELOPE code, the data from Bancheri are based on the EGSnrc code. As can be seen in figure 6, there are significant discrepancies between the different data sets. While the Monte Carlo data from this study, which were calculated in two different institutes, agree within 0.2%, the corresponding values from Bancheri for all chambers in question are about 1% smaller. On the other hand, the data from Bancheri are in good agreement with the Monte Carlo based data from Burns et al (2017), which were determined with the PENELOPE code. The available experimental data are again significantly smaller than all Monte Carlo based data sets, the deviations from the data of this study are already in the range of 2%, the deviations from the Monte Carlo data from Bancheri are in the range of 1%.
The difference between our Monte Carlo data and those of Bancheri is surprising, since the same code (EGSnrc) has been used and the transport parameters described by Bancheri do not obviously differ from those of this work. The only obvious differences are the use of the g code to calculate the air kerma Kair instead of the egs_kerma code and the use of different x-ray spectra. While CCRI-, H-and W-qualities were used in this work, TH-qualities were applied in Bancheri's work. At the moment, one can only speculate about the differences; one more reason could of course be different chamber models, but in both cases detailed blueprints from the manufacturer were used. Regarding the different user codes to calculate the air kerma free in air, a new version of the user code egs_kerma was recently published on GitHub (https://github.com/nrc-cnrc/EGSnrc/pull/610) including some bug fixes. It remains to be seen whether the new code can explain the differences between the calculated air kerma values. In any case, further research is necessary to clarify these differences.
Possible reasons for the discrepancies between the Monte Carlo data and experimental data are discussed in detail in the work of Bancheri et al (2020) and Burns et al (2017). According to this, these differences are due in particular to the ratio in the simulation and the measurement. According to the cited authors, it is currently not clear what these differences are due to.
Regarding the beam quality correction factors kQ,CCRI − 250 no comparable data are available in literature. All data presented here are given as a function of the beam quality specifier HVL (in mm Cu) and, as shown in figure 4, with one exception (PTW 31 010) all the data can be fitted by a simple logarithmic function. The quality of the fit is in all cases better than the fit for the ratios. The reason, why for the chamber PTW 31 010 a more bi-linear relationship with ln(HVL) occurs, is not yet clear. The only difference to the other PTW chambers is a smaller active volume.
The kQ,CCRI − 250 values for the same chamber, calculated with different Monte Carlo codes (EGSnrc, PENELOPE) agree at least on a 0.5% level. The agreement between the data from ENEA-INMRI and THM, both using the same Monte Carlo code, is even better. Regarding the different models of the IBA FC65 chamber the results show that even small deviations in the chamber geometry (10% change in the diameter of the central electrode) have a non-negligible impact on the resulting response of the chamber. This is a well known effect already described in literature (Ubrich et al
2008). From the comparison of figures 4–6 it can be concluded that the beam quality correction factor is more sensitive against changes in the geometry of the chamber then the perturbation factor pQ
. This is obviously due to the fact that
is a ratio of the chamber response of beam quality Q and Q0, whereas pQ
only includes data for the same beam quality Q.
5. Conclusion
In this study beam quality correction factors kQ,CCRI − 250 for absorbed dose to water based dosimetry protocols and perturbation factors pQ for air kerma based dosimetry protocols have been calculated for 14 different radiation qualities and six different ion chambers by Monte Carlo simulations. The calculations have been performed independently at three different institutions (IST, ENEA-INMRI and THM) using predefined reference conditions and transport parameters but different Monte Carlo codes (EGSnrc and PENELOPE). In all cases, the updated Monte Carlo cross sections according to the ICRU Report 90 were applied. The calculations performed by the partners for the same ion chamber models agreed in all cases within 0.5%.
The perturbation factors pQ calculated here differ from recently published literature values in the order of 1%, although the same Monte Carlo codes have been used. Compared to experimental pQ data, the values calculated here are even around 2% higher. The origin of these discrepancies is currently not clear and requires further investigation.
Acknowledgments
The authors would like to thank the companies PTW-Freiburg and IBA for providing detailed blueprints of the investigated ion chambers. The study was funded by the European Metrology Program for Innovation and Research (EMPIR) from the European Community, grant 16NRM03 'kQ -factors in modern external beam radiotherapy applications to update IAEA TRS-398'. The computing resources and the related technical support used for this work at ENEA-INMRI have been provided by CRESCO/ENEA-INMRIGRID High Performance Computing infrastructure and its staff (see http://www.cresco.enea.it for information).
Appendix A.: Numerical data
This appendix summarizes the essential Monte Carlo based data presented in the figures in numerical form. To be comparable with older publications (Büermann et al
2016) the data include not only the half-value layer but also the kerma weighted mean energy as beam quality specifier.
is defined as follows:

with , the photon fluence differential in energy free in air at a distance of 100 cm from the x-ray source.
Table A1. HVL, kerma weighted mean energy and beam quality correction factor kQ,CCRI − 250 according to equation (2) for the chambers NE2571, PTW30013. The number in parenthesis give the Type-A standard uncertainty of the Monte Carlo data of the last digits.
HVL |
![]() | NE 2571 | PTW 30 013 | ||||
---|---|---|---|---|---|---|---|
Spectrum | in mm Cu | in keV | IST | ENEA-INMRI | THM | ENEA-INMRI | THM |
CCRI-100 | 0.1461 | 44.5 | 0.963(2) | 0.9665(10) | 0.9649(6) | 0.9537(7) | 0.9534(6) |
CCRI-135 | 0.4708 | 66.8 | 0.983(2) | 0.9847(10) | 0.9848(6) | 0.9754(8) | 0.9738(6) |
CCRI-180 | 0.9863 | 91.6 | 0.991(2) | 0.9941(10) | 0.9937(6) | 0.9857(8) | 0.9856(6) |
CCRI-250 | 2.515 | 141.1 | 1.000(2) | 1.0000(10) | 1.0000(6) | 1.0000(7) | 1.0000(6) |
H-200 | 1.648 | 112.8 | 0.996(2) | 0.9980(10) | 0.9982(6) | 0.9948(8) | 0.9934(6) |
H-250 | 2.504 | 141.0 | 0.995(2) | 1.0002(10) | 0.9993(6) | 1.0005(7) | 0.9986(6) |
W-150 | 1.869 | 109.9 | 1.0001(9) | 0.9987(6) | 0.9972(8) | 0.9974(6) | |
W-200 | 3.125 | 146.3 | 1.0020(9) | 1.0022(6) | 1.0049(7) | 1.0028(6) | |
X-1 | 0.1062 | 41.3 | 0.9582(6) | 0.9472(6) | |||
X-2 | 0.1809 | 48.1 | 0.9692(6) | 0.9570(6) | |||
X-3 | 0.2485 | 52.7 | 0.9758(6) | 0.9625(6) | |||
X-4 | 0.3075 | 56.1 | 0.9793(6) | 0.9660(6) | |||
X-5 | 0.3587 | 58.7 | 0.9816(6) | 0.9686(6) | |||
X-6 | 0.403 | 61.9 | 0.9836(6) | 0.9711(6) |
Table A2. HVL, kerma weighted mean energy and beam quality correction factor
according to equation (2) for the chambers IBA FC65, PTW 31 013 and PTW 31 010. The number in parenthesis give the Type-A standard uncertainty of the Monte Carlo data of the last digits. Regarding the IBA FC65 chamber two different models were calculated by ENEA-INMRI and THM: FC65-G by ENEA-INMRI and FC65-GX by THM. Both models differ by the diameter of the central electrode. The PTW chambers 31 013 and 31 010 were only calculated by THM.
HVL |
![]() | IBA FC65 | ||||
---|---|---|---|---|---|---|
Spectrum | in mm Cu | in keV | ENEA-INMRI (type G) | THM (type GX) | PTW 31 013 | PTW 31 010 |
CCRI-100 | 0.1461 | 44.5 | 0.9725(9) | 0.9565(7) | 0.9628(7) | 0.9823(10) |
CCRI-135 | 0.4708 | 66.8 | 0.9901(9) | 0.9779(6) | 0.9787(7) | 0.9856(10) |
CCRI-180 | 0.9863 | 91.6 | 0.9951(9) | 0.9900(5) | 0.9882(7) | 0.9903(10) |
CCRI-250 | 2.515 | 141.1 | 1.0000(9) | 1.0000(5) | 1.0000(6) | 1.0000(10) |
H-200 | 1.648 | 112.8 | 0.9985(9) | 0.9961(5) | 0.9953(7) | 0.9945(10) |
H-250 | 2.504 | 141.0 | 1.0005(9) | 1.0007(5) | 1.0002(6) | 0.9989(10) |
W-150 | 1.869 | 109.9 | 0.9999(9) | 0.9990(5) | 0.9977(7) | 0.9966(10) |
W-200 | 3.125 | 146.3 | 1.0006(9) | 1.0032(5) | 1.0035(6) | 1.0021(10) |
X-1 | 0.1062 | 41.3 | 0.9509(6) | 0.9582(7) | 0.9804(10) | |
X-2 | 0.1809 | 48.1 | 0.9616(6) | 0.9653(7) | 0.9838(10) | |
X-3 | 0.2485 | 52.7 | 0.9664(6) | 0.9719(7) | 0.9839(10) | |
X-4 | 0.3075 | 56.1 | 0.9709(6) | 0.9731(7) | 0.9842(10) | |
X-5 | 0.3587 | 58.7 | 0.9740(5) | 0.9755(7) | 0.9847(10) | |
X-6 | 0.403 | 61.9 | 0.9759(5) | 0.9769(7) | 0.9854(10) |
Table A3. HVL, kerma weighted mean energy and perturbation factor pQ
according to equation (6) for the chambers PTW 31 013 and NE 2571. The number in parenthesis give the Type-A standard uncertainty of the Monte Carlo data of the last digits.
HVL |
![]() | PTW 30 013 | NE 2571 | |||
---|---|---|---|---|---|---|
spectrum | in mm Cu | in keV | ENEA-INMRI | THM | ENEA-INMRI | THM |
CCRI-100 | 0.1461 | 44.5 | 1.0139(9) | 1.0154(7) | 1.0126(10) | 1.009(7) |
CCRI-135 | 0.4708 | 66.8 | 1.0291(9) | 1.0287(7) | 1.0293(10) | 1.0306(7) |
CCRI-180 | 0.9863 | 91.6 | 1.0265(9) | 1.0253(7) | 1.0314(10) | 1.0305(7) |
CCRI-250 | 2.515 | 141.1 | 1.0168(9) | 1.0150(7) | 1.0203(10) | 1.0183(7) |
H-200 | 1.648 | 112.8 | 1.0221(9) | 1.0204(7) | 1.0270(10) | 1.0256(7) |
H-250 | 2.504 | 141.0 | 1.0166(9) | 1.0149(7) | 1.0210(10) | 1.0178(7) |
W-150 | 1.869 | 109.9 | 1.0218(9) | 1.0213(7) | 1.0273(10) | 1.0241(7) |
W-200 | 3.125 | 146.3 | 1.0149(9) | 1.0120(7) | 1.0172(10) | 1.0147(7) |
X-1 | 0.1062 | 41.3 | 0.9996(7) | 0.9906(7) | ||
X-2 | 0.1809 | 48.1 | 1.0157(7) | 1.0138(7) | ||
X-3 | 0.2485 | 52.7 | 1.0238(7) | 1.0229(7) | ||
X-4 | 0.3075 | 56.1 | 1.0276(7) | 1.0273(7) | ||
X-5 | 0.3587 | 58.7 | 1.0284(7) | |||
X-6 | 0.403 | 61.9 | 1.0291(7) | 1.0321(7) |
Table A4. HVL, kerma weighted mean energy and perturbation factor pQ
according to equation (6) for the chambers IBA FC65, PTW 31 013 and PTW 31 010. The number in parenthesis give the Type-A standard uncertainty of the Monte Carlo data of the last digits. Regarding the IBA FC65 chamber two different models were calculated by ENEA-INMRI and THM: FC65-G by ENEA-INMRI and FC65-GX by THM. Both models differ by the diameter of the central electrode. The PTW chambers 31 013 and 31 010 were only calculated by THM.
HVL |
![]() | IBA FC65 | ||||
---|---|---|---|---|---|---|
Spectrum | in mm Cu | in keV | ENEA-INMRI (type G) | THM (type GX) | PTW 31 013 | PTW 31 010 |
CCRI-100 | 0.1461 | 44.5 | 1.0082(10) | 1.0080(7) | 1.0105(8) | 1.0205(12) |
CCRI-135 | 0.4708 | 66.8 | 1.0297(10) | 1.0231(7) | 1.0255(8) | 1.0380(12) |
CCRI-180 | 0.9863 | 91.6 | 1.0287(10) | 1.0248(7) | 1.0247(8) | 1.0333(12) |
CCRI-250 | 2.515 | 141.1 | 1.0206(10) | 1.0148(7) | 1.0129(7) | 1.0164(11) |
H-200 | 1.648 | 112.8 | 1.0259(10) | 1.0214(7) | 1.0188(8) | 1.0235(12) |
H-250 | 2.504 | 141.0 | 1.0209(10) | 1.0165(7) | 1.0136(8) | 1.0154(12) |
W-150 | 1.869 | 109.9 | 1.0259(10) | 1.0211(7) | 1.0173(8) | 1.0222(12) |
W-200 | 3.125 | 146.3 | 1.0168(10) | 1.0133(7) | 1.0080(7) | 1.0103(12) |
X-1 | 0.1062 | 41.3 | 0.9946(7) | 0.9948(8) | 1.0038(12) | |
X-2 | 0.1809 | 48.1 | 1.0116(7) | 1.0119(8) | 1.0247(12) | |
X-3 | 0.2485 | 52.7 | 1.0182(7) | 1.0219(8) | 1.0319(12) | |
X-4 | 0.3075 | 56.1 | 1.0219(7) | 1.0237(8) | 1.0345(12) | |
X-5 | 0.3587 | 58.7 | 1.0234(7) | 1.0270(8) | 1.0402(12) | |
X-6 | 0.403 | 61.9 | 1.0244(7) | 1.0278(8) | 1.0398(12) |
Footnotes
- 6
See: http://www.rtnorm.eu.